RADIOMETER
The tcpCO2 handbook 2011
Handbook
101 Pages
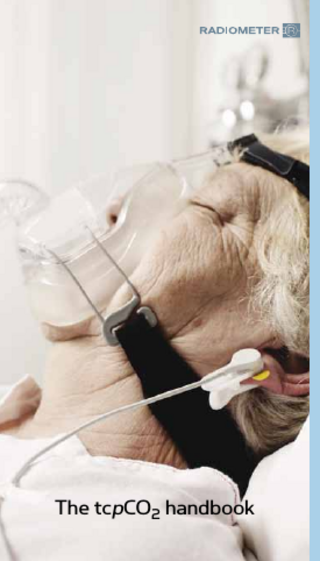
Preview
Page 1
Author: Scientific Advisor Annette Melhedegaard Thomsen, Radiometer Medical ApS. Contributor: Prof. Dr. Konrad E. Bloch, Universitäts Spital Zürich. With input from Kevin Bage, Radiometer LTD, Franz von Wirth, Radiometer GmbH and Phil Lazzara, Radiometer America Inc. Copyright © 2012 Radiometer Medical ApS, Denmark. Contents may be reproduced if the source is acknowledged. Printed in Denmark by Radiometer Medical ApS, Åkandevej 21, 2700 Brønshøj, Denmark, 2011. ISBN 978-87-91026-09-6 939-049. 201201E.
0459 Data subject to change without notice. Radiometer, the Radiometer logo, ABL, AQT, TCM, RADIANCE, AQURE, PICO, CLINITUBES and QUALICHECK are trademarks of Radiometer Medical ApS.
Introduction to transcutaneous monitoring tcpCO²/tcpO² monitoring... 6 TC methodology... 10 Combined measurements of tcpCO² SpO²... 12 General TC application advice... 20 Choice of measuring sites... 26 Choice of measuring temperature... 29
Transcutaneous monitoring applications Neonatology... 33 Pediatrics... 40 Operating room, ICU and step-down units ... 45 Conscious sedation during diagnostic surgery... 50 Pulmonary/respiratory medicine laboratories or wards... 54 Sleep laboratories... 62 Veterinary hospitals/laboratories... 66
Use of TCM4/40/TOSCA/CombiM monitors Menu structure of TCM4/40/TOSCA/CombiM monitors... 70 Short-form support for: The TCM4/40/TOSCA/CombiM monitors... 71 How to apply TC sensor... 78 Calibration ... 81 Cleaning... 84 Maintenance of the sensor... 86 List of abbreviations ... 93
Complementary techniques Choice of sensor site and temperature... 101
Introduction to transcutaneous monitoring
4
The transcutaneous carbon dioxide and oxygen measuring techniques were first developed in the nineteen seventies. Since then, major further advances have been made and significantly improved the technology and practical application. Today, monitoring of transcutaneous gas tension is not only valuable for the respiratory and ventilatory status of neonates, but also highly relevant for the ventilatory care of children and adults with chronic respiratory failure. This development has followed the change in hospital care. Trends go in two directions: towards an increased application of non-invasive acute and long-term ventilation, and towards a more frequent use of techniques like high-frequency jet ventilation (HFJV), highfrequency oscillatory ventilation (HFOV) and other novel acute care therapeutic modalities. Together, these changes have led to an increased need for continuous focus on the patient’s carbon dioxide (CO2) status. Transcutaneous CO2 monitoring meets these requirements non-invasively. This handbook is primarily a guide to transcutaneous carbon dioxide (tcpCO2) monitoring from a clinical perspective. However, as transcutaneous oxygen (tcpO2) monitoring is sometimes relevant for the same patients (e.g. neonates on oxygen), the handbook also contains information on this parameter. For more in-depth information on tcpO2 monitoring of patients with peripheral arterial disease (PAD), e.g. critical ischemia, please refer to “The tcpO2 handbook”. [*]
* Thomsen A, Wirth FV, Bryde-Jacobsen J. The tcpO2 handbook. Radiometer Medical ApS, Åkandevej 21, 2700 Brønshøj, Denmark, 2003.
As measurement of oxygen saturation by pulse oximetry (SpO2) is often used in combination with tcpCO2, and sometimes also tcpO2 monitoring, this technique is described as well. The handbook is based on scientific literature and the operator’s manuals of Radiometer TCM monitors. According to the findings in clinical studies, the handbook provides suggestions on patient categories for which TC monitoring can be used. References are found at the end of each section. For a more detailed presentation of technical issues and troubleshooting of transcutaneous monitors, please refer to the operator’s manuals of these products. Each section contains blank pages for the reader to make relevant notes and modifications according to local policies and procedures. Note: The validity of any measurement must be carefully examined by a clinician/healthcare professional and related to the patient’s clinical condition before any decisions are made on the basis of the measurement.
5
tcpCO2/tcpO2 monitoring Continuous non-invasive monitoring Patients with respiratory and cardiovascular disease, during anesthesia and surgery depend on having their carbon dioxide and oxygen status surveyed. Hypercapnia and hypoxia can result in acidosis requiring treatment; if undetected hypercapnia and hypoxia may lead to acidosic, hyperkalemia, myocardial depression, arrhytmias, arterial hypotension or hypertension, intercranial hypertension, narcosis and organ damage. Hypocapnia and hyperoxia may also have adverse and even severe damaging effects on the brain and other organs due to the vulnerability of cells and organs to changes in blood pH level and their dependence on oxygen. Continuous non-invasive transcutaneous monitoring of carbon dioxide and oxygen may be obtained in various ways and combinations: 6
• tcpCO2 • tcpO2 • SpO2 • tcpCO2 + tcpO2 • tcpCO2 + SpO2 • tcpCO2 + tcpO2 + SpO2
Transcutaneous measurement of carbon dioxide and oxygen Transcutaneous carbon dioxide and oxygen monitoring is a non-invasive way of continuously measuring the tension of these gases in the skin. A combined Clark-type and Severinghaus-type sensor also called Stow-Severinghaus sensor* is placed on the skin and heated. The sensor heat dilates the underlying capillaries and increases the gas diffusion through the lipid structure of the skin, thereby allowing carbon dioxide and oxygen to diffuse up to 20 times more quickly from the capillaries through the skin to the sensor. The oxygen generates a current and the carbon dioxide a potential in the sensor. These signals are converted by the monitor and showed as tcpCO2 and tcpO2 values on the screen. 7
*In the literature the pCO2 sensor is generally known as a Severinghaus-type sensor. However, Richard Stow originally invented the gas measuring technique and described it in 1954. Later that year, Leland C. Clark Jr. designed an arterial blood gas pO2 sensor. In 1958 John W. Severinghaus developed a complete blood gas apparatus by combining his more practical version of Stow’s pCO2 sensor with Clark’s pO2 sensor. This is the reason why; the pCO2 sensor is also known by the name Stow-Severinghaus sensor. Dietrich Lübbers, Albert and Renata Huch demonstrated together with Patrich Eberhard in 1972 that heating the skin to 42-45° C creates vasodilatation, which makes the cutaneous surface pO2 value reasonable close to PaO2, especially in newborn babies. After that were the transcutaneous pCO2 sensor also developed and introduced to the market in the early 1980s [1].
The sensor on the skin
CO2
O2
O2
O2 Arterial end of a capillary loop
CO2
O2
CO2
CO2
O2
O2
O2
O2
CO2 CO2
8
Venous end of a capillary loop
CO2
FIG. 1: Transcutaneous pCO2/O2 monitoring (TC) The heat from the sensor dilates the capillary and increases local blood flow and the diffusion of CO2/O2 through the skin to the sensor. tcpCO2/tcpO2 is measured electrochemically inside the sensor.
Are tcpCO2/tcpO2 identical to arterial blood gases? It is a misconception that tcpCO2/tcpO2 are the same as arterial blood gases. The purpose of monitoring transcutaneous gas tensions is to obtain information on the cardio-respiratory condition of a patient without the need to repeatedly draw arterial blood samples for analysis. However, the transcutaneous partial pressure of oxygen reflects the underlying dermal pO2 level that is influenced not only by the arterial pO2 but depends on local blood flow, oxygen release from hemoglobin and skin metabolism. Living skin will always consume some oxygen and tcpO2 will therefore always be lower than the arterial pO2 irrespective of the sensor measuring temperature. Similarly, dermal pCO2 measured by a transcutaneous sen-
Heat Oxygen Metabolisme
Carbon dioxide production
O2: 92 mmHg
CO2: 59 mmHg
O2: 145 mmHg
CO2: 55 mmHg
O2: 100 mmHg
CO2: 40 mmHg
FIG. 2: Example of the different levels of gases in blood to tissue Illustration of the physiological oxygen and carbon dioxide contents in a healthy subject’s arterioles, capillaries and skin cells, when the heat from the TC sensor dilates the capillary and increases local blood flow. As part of the metabolic reaction there will be some oxygen consumption and an additional carbon dioxide production.
sor is not only determined by the arterial PaCO2 but also influenced by local blood flow, and the skin metabolism. The cells metabolic´s pCO2 addition is temperature-dependent, this influence is minimized by applying a temperature-specific constant and a metabolic factor; however, there will still be some difference between the tcpCO2 value and the carbon dioxide tension in the arterial blood. Generally tcpCO2 would be higher than PaCO2 [2,3]. tcpCO2/tcpO2 measures skin pCO2/pO2 tcpCO2/tcpO2 monitoring is an easy and non-invasive method that reflects the trends of changes of arterial blood gases. It provides assessment of the tissue’s oxygenation and carbon dioxide removal via the cardio-pulmonary system. This information can indicate if an arterial puncture is required [4].
9
TC Methodology Measurement of carbon dioxide tension tcpCO2 measurement is in fact a pH measurement. pCO2 from the skin diffuses through the membrane of the sensor into an electrolyte solution. Here it reacts with water, forming carbonic acid that immediately dissociates into HCO3- and H+ according to the following equation: H2O + CO2DH2CO3DH+ + HCO3-
10
As HCO3- in the electrolyte solution is kept at a fixed level, changes in the H+ concentration will always be equivalent to changes in pH. These pH changes alter the voltage between the glass sensor and the reference sensor. The measured pH is converted into a carbon dioxide reading by the HendersonHasselbalch equation and is shown as a tcpCO2 value in either mmHg or kPa [5,6] Oxygen measurement Oxygen from the skin passes through the sensor membrane. It reaches the cathode, which consists of platinum. Here oxygen is reduced as a result of the current-generating process: O2 + 2H2O + 4e- → 4OHThe silver ring surrounding the platinum cathode is the anode where the following oxidation reaction takes place: 4Ag + 4CL- → 4AgCl + 4eThe reduction of oxygen generates a current that is converted by the monitor into a voltage and a digitized tcpO2 value in mmHg or kPa [5,6].
Notes
11
1. 2.
3.
4. 5. 6.
Severinghaus JW, Astrup P, and Murray JF. Blood gas analysis and critical care medicine. Am J Respir Crit Care Med. 1998; vol. 157; nr. 4: 114-22. Wimberley PD, Frederiksen PS, Witt-Hansen J. et al. Evaluation of a transcutaneous oxygen and carbon dioxide monitor in a neonatal intensive care department. Acta Paediatr Scand 1985; 74: 352-59. Wimberley PD, Pedersen KG, Olsson J. et al. Transcutaneous carbon dioxide and oxygen tension measured at different temperatures in healthy adults. Clin Chem 1985; 31, 10: 1611-15. Hill KM, Klein DG. Transcutaneous carbon dioxide monitoring. Crit Care Nurs Clin N Am 2006; 18: 211-15. Larsen J, Linnet N. tc 110: Solid state transcutaneous combined pO2/pCO2 sensor. Radiometer A/S, Denmark 1990 (ISBN 87-88138-283) TOSCA 500 Operating Manual. Radiometer Basel AG, Radiometer Medical ApS. 2006; Publ. No: 520.81001/3 – issued Jan. Part No.: 520 0910
Combined measurements of tcpCO2 and SpO2 Combined tcpCO2 and SpO2 sensor Pulse oximetry provides instant and continuous information about oxygen saturation and pulse rate. It does not, however, indicate how much hemoglobin is available in the blood. Oxygen saturation measurement is often used together with tcpCO2 as they compliment each other and provide a wider perspective of the patient’s blood gas status. Pulse oximetry is widely accepted because of its non-invasive character, ease of use and low cost. It is also considered easy to interpret; however, the technique has certain limitations that are important to keep in mind in order to obtain optimum patient monitoring.
12
SpO2 methodology A pulse oximeter sensor typically contains two light-emitting diodes (LEDs) – one with red light and one with infrared light. The light passes through a blood-perfused tissue to a photodetector on the other side of the finger, earlobe, foot or toe or is reflected by bone and other structures. The more lightabsorbing blood that is present at the moment of measurement, the less light reaches the photodetector [7]. The illustration next page shows a spectrophotometric measurement of blood with an overlap of the red (660 nm) and infrared (900 nm) light. The two absorption peaks represent oxygenated (O2Hb) and deoxygenated (HHb) hemoglobin. Because O2Hb absorbs less red light than infrared light, the underlying blood flow at high saturation values has less influence on the detected red signal than on the infrared one. At
low saturation values this situation is reversed and far more red than infrared light is absorbed. Thus, the arterial oxygen saturation can be derived from the ratio of the red and infrared light absorption. SpO2 measurements indicate how much oxygen is actually bound to the available hemoglobin in the blood, but it does not show the hemoglobin or dyshemoglobin concentration. Therefore SpO2 might not always show the actual blood oxygen status [8].
Red LED Spectrum
IR LED Spectrum
13
600
700
800
900
1000
Wavelength nm FIG. 3: LED-emitted light spectrum Overlapping of typical LED-emitted light spectra and relative light absorption spectra of oxygenated and deoxygenated hemoglobin. The dashed purple line indicates the spectrum of 50 % saturated blood, with the relative absorbance in the red and infrared indicated by the black circles. Figure and text is reprinted by permission of Nellcor Puritan Bennett Inc. Pleasanton, California [8].
The relation between SpO2 and pO2 The oxygen dissociation curve describes the relationship between oxygen tension (pO2) and oxygen saturation (SpO2) at standard conditions (Fig. 4). This relationship can be used with some restriction to estimate pO2 from SpO2. The position of the dissociation curve is, however, shifted either to the left or the right by changes in pH, temperature, 2,3-DPG, pCO2 level, and the presence of dyshemoglobins.
14
A multicenter study of these variables resulted in the curves shown in (Fig. 5). When oxygen saturation is read from this curve with a PaO2 of 8 kPa (60 mmHg), the corresponding SpO2 value ranges from 69.7 % to 99.4 %. At SpO2 level of 90 %, the PaO2 values ranges were 3.82 and 18.3 kPa (29 and 137 mmHg). Beside this inaccuracy in some patients, it is also important to realize that, SpO2 cannot differentiate between hypoxemia caused by ventilation/perfusion mismatch and that caused by alveolar hypoventilation. These examples illustrate that oxygen saturation does not always provide a precise estimate of hypo- and hyperoxia. In terms of oxygen transport, however, the oxygen saturation technique together with the hemoglobin measurements is quite important [9,10].
sO2 0.9 0.8 0.7 0.6 0.5 0.4 0.3
p50
0.2 0.1
20 0
2
4
40
60
6
8
pO2 mmHg
80 10
12 kPa
FIG. 4: Oxygen tension vs. saturation The Oxygen Dissociation Curve. p50 is defined as the oxygen tension of half saturation, sO2 = 50 %
sO2 1.0
15
0.9 0.8 0.7 0.6 0.5 0.4 0.3 0.2 0.1 0.0
0
5
10
15
20
25
pO2kPa
FIG. 5: Oxygen tension vs. saturation The curve from a multicenter study shows the measured oxygen tension vs. the saturation in 10,079 arterial blood samples [9].
SpO2 bias due to motion, level of skin pigmentation Motion artifact is often a problem for pulse oximetry and may result in false readings or alarms. A study that compared finger saturation probes and the number of false alarms showed significantly fewer false alarms on the middle finger compared with measurements on the index finger, which is most often used as measuring site. SpO2 measurements may also exhibit erroneous readings due to high patient skin pigmentation or nail polish. Some sensors may even show a small bias when measuring on women. Furthermore, correct sensor fixation is very important to ensure optimum light-tissue interaction and thereby reliable measurements [11,12,].
16
Earlobe sensors vs. finger SpO2 sensors A study showed that a combined earlobe tcpCO2/SpO2 sensor detects changes in oxygen saturation significantly earlier than a finger SpO2 sensor. This can be explained by a difference in the lung-to-ear vs. lung-to-finger circulation time and by differences in the vascularization of the monitored tissue. The signal processing technique, and the use of a heated sensor affect the response time of pulse oximetry as well. Other studies have shown that the earlobe is less affected by systemic vasoconstriction, which also makes the earlobe a good site for transcutaneous carbon dioxide measurement [13,14,15,16,17].
100 90 80 70 18 O2 %/min
60
28 O2 %/min 1min
50
Combined tcpCO2/SpO2 SpO2 Standard finger FIG. 6: SpO2 response time The response time of a heated combined tcpCO2/SpO2 earlobe sensor is faster than that of an unheated finger probe. As a result, the detection of the beginning and end of the desaturation happens 20-30 seconds earlier by the earlobe sensor and the obtained amplitude is nearly two times greater, indicating a larger specificity. This is important for patients with rapid and transient changes in SpO2 for example sleep apnea. As not only the number of desaturation events but also the degree of these events correlate with the impairment of the cognitive function and with cardiovascular complications. A short response time is essential in order to detect fast changes of the oxygen saturation [15].
17
The combined tcpCO2/SpO2 sensor The combined tcpCO2/SpO2 sensor pictured below gives fast information of these parameters together with the pulse rate. LEDs
photodiode
arterialized capillaries arteries ear lobe vens attachment clip
FIG. 7: The tcpCO2/SpO2 sensor tcpCO2/SpO2 is measured by an earlobe sensor attached by a clip. The technique is a combination of the above shown TC technique and a spectrophotometric pulse oximetry measurment. This TC sensor contains a gold shield that protects the membrane, thereby allowing the sensor to provide reliable measurements for up to 14 days without membrane change. Recommended measuring temperature: 42 °C (108 °F).
18
SpO2 is widely accepted due to the non-invasive character of the method, its ease of use and low costs – and when the limitations of the oxygen saturation technique are taken into consideration, the results are easy to interpret. SpO2 measurement in combination with tcpCO2 provides a continuous estimation of the patient’s arterial oxygen saturation and of pulse rate together with information on the carbon dioxide tension in the skin and thereby the body´s ability to oxygen uptake and to eliminate carbon dioxide via the cardiopulmonay system. All together this provides valuable information of the patient’s cardio-respiratory status as an adjunct to decision making on the patient’s care.
Notes
7.
8. 9.
10. 11.
12. 13.
14.
15.
16.
17.
Haymond S. Oxygen saturation; a guide to laboratory assessment. Clinical Laboratory News 2006; Feb: 10-12. Reprint on the AACC website: www. aacc.org; Clinical Laboratory News; Series Articles. Nellcor Puritan Bennett Inc. A technology overview of the Nellcor oximax pulse oximetry system. Web information. Gøthgen IH, Siggaard-Andersen O, Kokholm G. Variations in the hemoglobin-oxygen dissociation curve in 10,079 arterial blood samples. Scand J Clin Invest 1990; 50, suppl. 203: 87-90. Poets CF. Pulse oximetry vs. transcutaneous monitoring in neonates: practical aspects. www.bloodgas.org 2003; October: Neonatology. Richards NM, Giuliano KK, Jones GJ. A prospective comparison of 3 newgeneration pulse oximetry devices during ambulation after open heart surgery. Respiratory Care 2006; 51, 1: 29-35. Poets CF, Stebbens VA: Detection of movement artefact in recorded pulse oximeter saturation. European Journal of Pediatrics 1997; 156, 10: 808-11. Hickson VM, Mathews GR. Pulse oximeter sensor accuracy. Poster presentation from the ISAMOV 2007 conference (15-17 Mars 2007 Duke University, Durham, North Carolina). Senn O, Clarenbach CF, Kaplan V et al. Monitoring carbon dioxide tension and arterial oxygen saturation by a single earlobe sensor in patients with critical illness or sleep apnea. Chest 2005; 128, 1291-96. Bloch KE. Tosca – selected case reports. Adipositas-hypoventilation syndrome. 2005; Patient 1: Figure 2: 5. (Linde Medical Sensors AG, Austrasse 25, Ch-4051 Basel, Switzerland) Jablonka DH, Aymen A, Stout RG. What is the optimum site to measure the effect of spontaneous ventilation on the pulse oximeters waveform? ASA annual meeting abstracts 2004; A-191. Awad AA, Stout RG, Ghobashy AM. Analysis of the ear pulse oximeter waveform. Journal of Clinical Monitoring and Computing 2006; 20, 3: 174-84.
19
General TC application advice Practical application An ideal TC measuring site is an area of skin over a homogeneous capillary bed with no large veins, skin defects or hair. Placing the sensor directly on top of a bone or a scar may cause erroneous results because of locally impaired perfusion of these sites. Severe edema may also lead to unreliable results because of the reduction of blood flow caused by a compression of the capillary loops and a longer diffusion pathway. For more information, refer to the section on measuring sites on page 26.
20
The sensor The sensor must be in contact with the skin through the contact liquid or gel. This is to avoid bias from atmospheric air between tissue and sensor. If there are air bubbles, they will affect the measurement, resulting in too high oxygen and too low carbon dioxide levels. For more information, refer to the section on how to apply the TC sensor on page 78. Initiation of TC monitoring involves sensor stabilization time It takes about 3-7 minutes after the sensor has been placed on the skin for the tcpCO2 values to stabilize and it may take a further 10-17 minutes to obtain reliable tcpO2 values. Preheating the skin may reduce this time to 3-6 and 5-16 minutes, respectively. tcpCO2/tcpO2 is considered stable when TC values during stable patient conditions do not change more than about ± 2 mmHg (±0.25 kPa) within 1 minute.
tcpCO2 lag and response time A study in healthy subjects evaluated tcpCO2 lag time and defined it as: “The time from the initiation of an intervention of the gas status to the initial response of the sensor”. The mean lag time for tcpCO2 during hypercapnia was 16.8 ± 1.3 seconds. When the subjects resumed breathing room air from hypercapnia, the mean lag time was 14.2 ± 1.4 seconds. The response time may be defined as: The initiation of an intervention in the gas status to the maximum (100 %) response of the sensor. The 90 % response time was 77.9 ± 6.7 seconds. tcpCO2 has a shorter response time compared with tcpO2. This is most likely due to a hypercapnia-induced increased cardiac output, capillary perfusion and faster carbon dioxide diffusion through the epidermis [18].
mmHg pCO2 80 70
90%
21 alveolar pCO2 tcpCO2
Log time
60 50 40 30 20 0
50
100
150
Lag time Response time FIG. 8: The tcpCO2 response and lag time
200
250
Time (sec.)